Overview
Our research uses theory and computation to understand the fundamental transport and reaction processes that occur in electrochemical systems relevant to energy storage and environmental applications, such as Li-ion batteries, electrochemical CO2 capture, and water treatment. We develop and integrate principles from transport phenomena, statistical mechanics, thermodynamics, kinetics, and solid-state physics to understand how disorder, in the form of chemical structural heterogeneities like chemical impurities, amorphous solid structures, and tortuous geometries, affects the fundamental electrochemical and transport mechanisms in these systems. Our aim is to develop quantitative theoretical and computational models that can accurately predict the behavior of these highly non-ideal systems, thereby providing insights and guidelines for the design of efficient electrochemical devices.
Philosophy and Methodologies
We believe that every research challenge is unique. Although various analytical and computational methodologies are available to address many problems, we often find that new approaches are necessary to tackle unanswered research questions. Thus, we follow the research philosophy of pursuing questions that excite us without being constrained by the tools at our disposal, enabling us to develop innovative analytical and computational methodologies and thus push our understanding of complex phenomena.
In our group, we draw inspiration from theoretical methodologies developed in fields such as non-equilibrium thermodynamics, stochastic processes, statistical mechanics, condensed matter physics, quantum dynamics, and dynamical systems. We use these methodologies to analytically address research challenges and develop theoretical models with quantitative accuracy. Additionally, we develop and use computational methodologies for continuum and molecular systems, as well as combinations of these, to support our theoretical models and provide quantitative comparisons with experiments. Examples of computational methodologies we have developed and used include finite element and spectral methods, molecular dynamics and Monte Carlo algorithms, and enhanced sampling techniques.
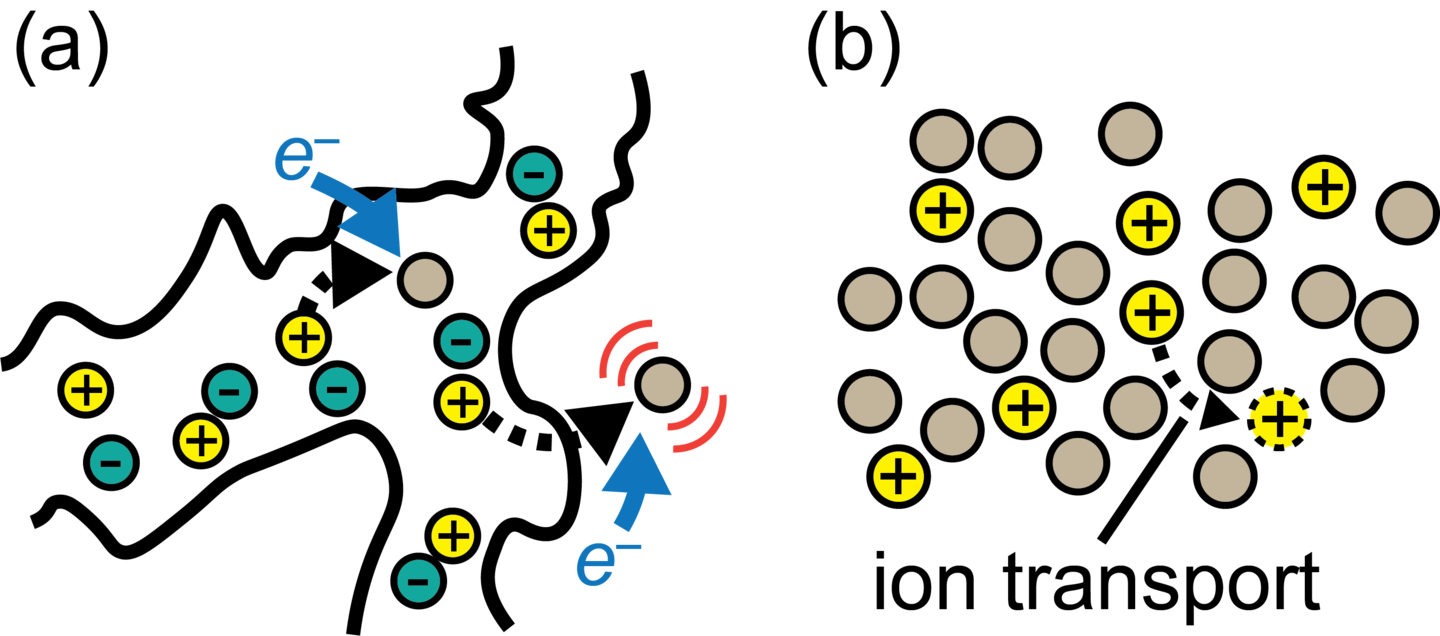
Thermodynamics and Transport of Ions in the Micro- and Meso-scale
We are interested in understanding how thermodynamics and transport properties are affected by the existence of disorder and heterogeneities associated with length scales ranging from a couple of ions (nm) to typical distances between active particles within porous electrodes (μm). We develop theories based on a combination of statistical mechanics, electrostatics, and transport phenomena, which allow us to quantitatively predict ionic activity coefficients, diffusivities, and conductivities. These predictions are then used to assess the performance of electrochemical systems associated with energy storage (Li-ion batteries) and environmental applications (CO2 capture and water treatment). In addition, we develop molecular and continuum-level simulation methodologies and models that can provide direct comparisons to experiments.
Reaction and Transport Mechanisms at Electrochemical Interfaces
The performance of electrochemical systems is closely tied to the electrochemical activity at their electrodes. We are interested in revealing how RedOx and electrodeposition reactions are influenced by the thermodynamic and transport properties of the species and phases involved in these processes, as well as the effects of electrode morphology on electrochemical reaction activity. Through theoretical models of deposition and RedOx reactions, combined with the thermodynamics of material discontinuities (e.g., electrolyte/electrode interfaces), and molecular and continuum computational methodologies, we provide quantitative predictions on the electrochemical behavior of interfaces relevant to advanced energy storage technologies (e.g., Li metal and other multivalent metal batteries).
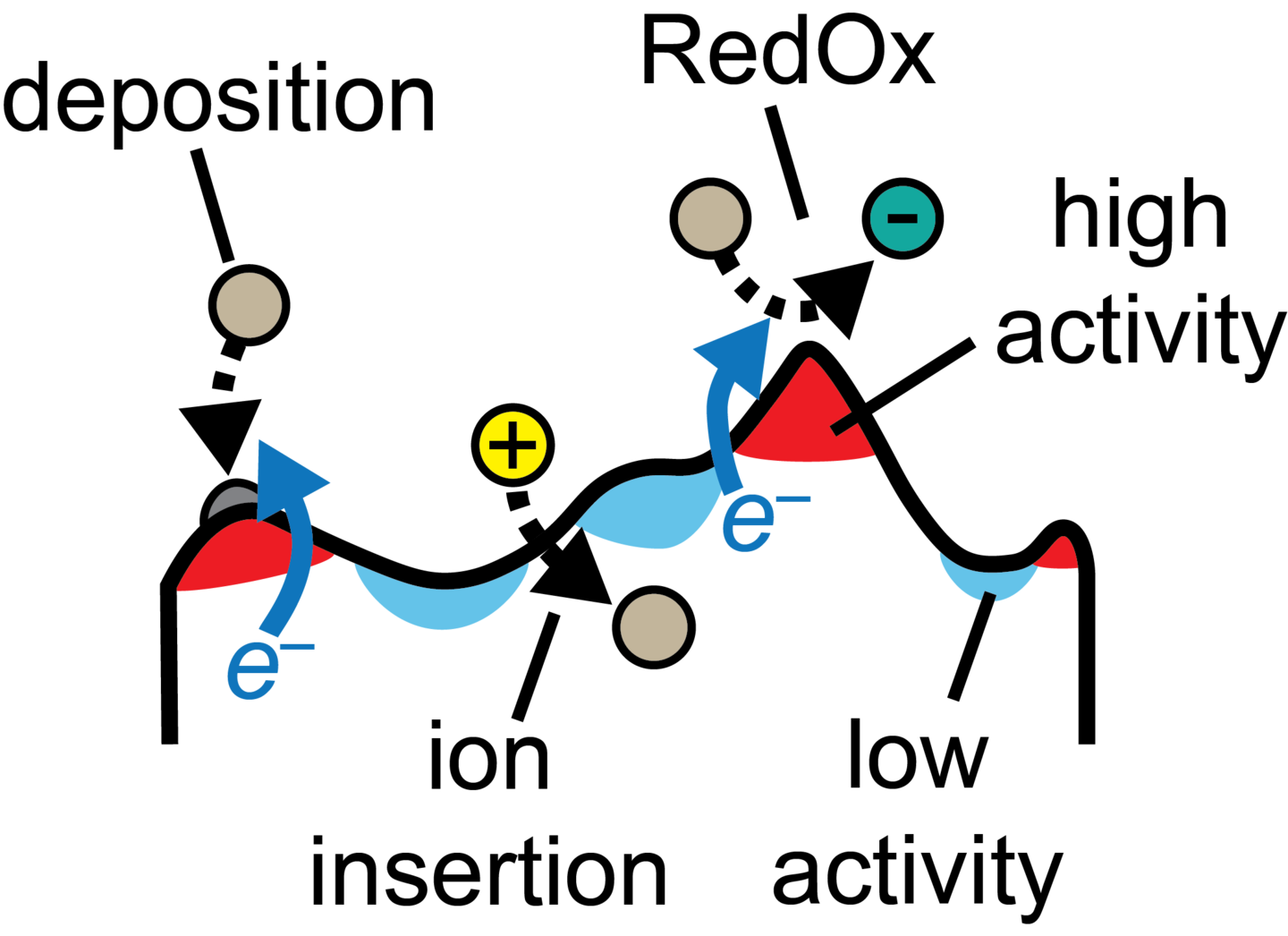
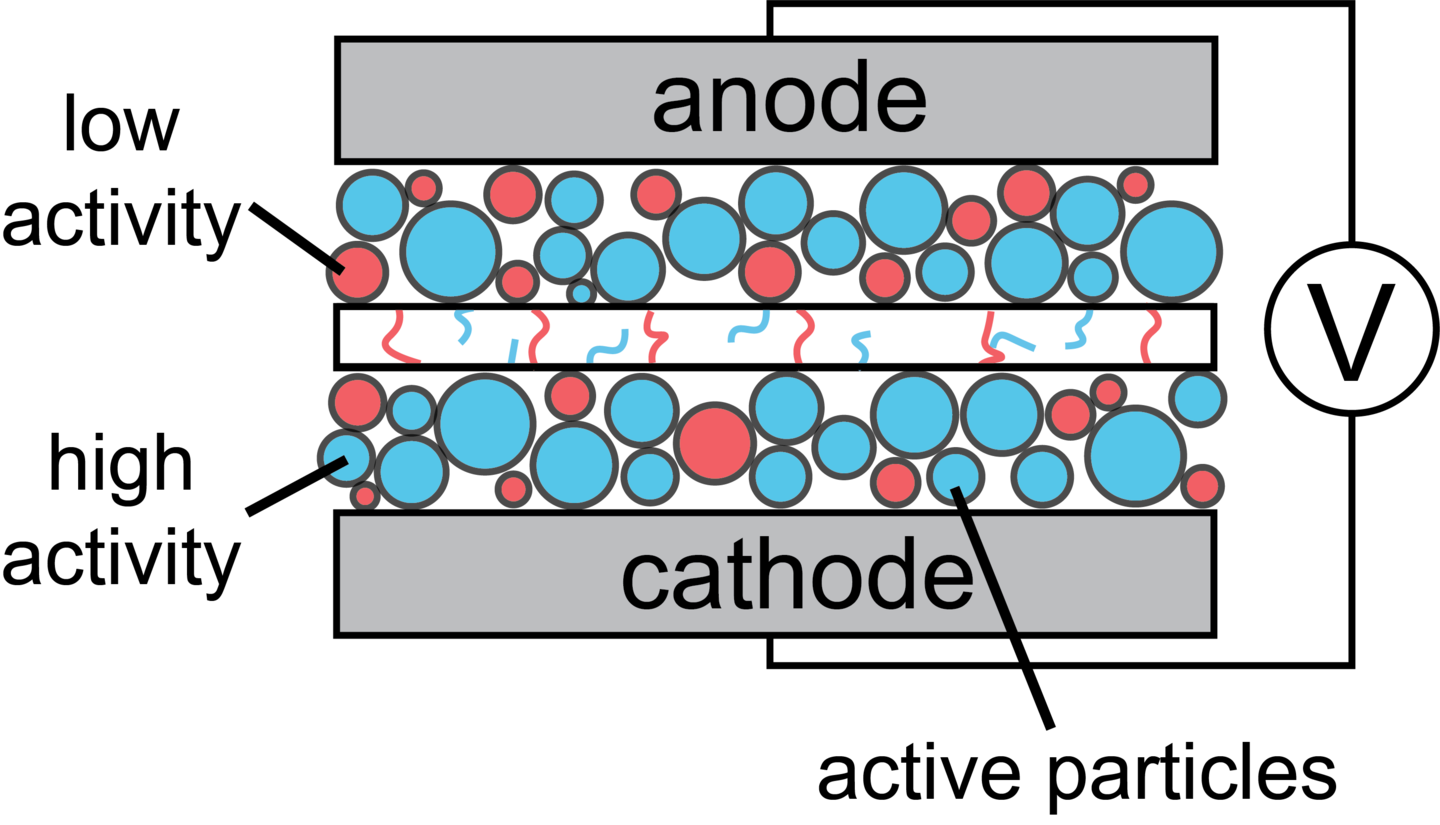
Electrochemical Activity in Porous Structures
Most electrochemical devices are composed of a collection of active particles where electrochemical reactions occur, tortuous pores that facilitate ion transport, and an electronically conductive network for electron flow. The varying shapes and sizes of active particles and tortuous pores, along with the heterogeneously distributed conductive network, result in spatially and temporally varying electrochemical activity during device operation. We aim to understand the fundamental mechanisms causing electrochemical heterogeneity across porous electrodes by developing theories inspired by the statistical mechanics of spin and molecular glasses, combined with electrochemical kinetics and transport phenomena fundamentals. Our goal is to quantitatively predict the degree of electrochemical activity heterogeneities, identify the factors contributing to this behavior, and determine how to exploit it for the design of optimally operating electrochemical devices. Additionally, we develop coarse-grained computational models that describe the emergent electrochemical behavior at the device level, providing insights into the operational protocols required for efficient device operation.